1 INTRODUCTION AND HISTORICAL OVERVIEW
The aminobenzyl penicillin, amoxicillin, was introduced in 1972 to succeed the earlier ampicillin, which was introduced in 1961. On its own or in combination with the beta-lactamase inhibitor clavulanic acid, which was introduced in 1982, amoxicillin has formed the backbone of antibiotic therapy in both human and veterinary medicine for many common infections and has also been widely used in swine medicine over the years. There are a number of formulations available, injectables (both short-acting (SA) and long-acting (LA) formulations), oral dosers for piglets, but these have been discontinued, soluble powders for water medication and also oral powders and feed premixes for use on/in feed. In 2006, the World Organisation for Animal Health (OIE, 2006) published a list of critically important antibiotics for veterinary use and the penicillins and beta-lactamase inhibitors were included, especially as they combined exceptional bactericidal efficacy with low toxicity, either alone or in combination. With the concerns over the extensive use of antibiotics in humans and animals and the increasing levels of resistance found and the possibilities that resistance may be transferred to human, governments are increasingly concerned about animal use and wish antibiotics to be used more responsibly and their volumes reduced. The World Health Organization (WHO, 2017) has classified aminopenicillins, like amoxicillin, as critically important antibiotics along with third- and fourth-generation (G) cephalosporins, which are largely resistant against the more basic beta-lactamases, the fluoroquinolones, aminoglycosides and macrolides. The European Medicines Agency (EMA, 2014) has currently classed amoxicillin alone and in combination with clavulanic acid as Category 2, a higher risk to public health, along with third- and fourth-G cephalosporins and fluoroquinolones but not macrolides, which are in Category 1, along with narrower spectrum penicillins and beta-lactamase resistant ones. Penicillins are meant to be reviewed in 2017 by the EMA. The Food and Drug Administration (FDA, 2003) in the United States of America (USA) has classed amoxicillin as highly important, along with the fourth-G cephalosporins, whereas the third-G cephalosporins, fluoroquinolones and macrolides were classed as critically important. There are some discrepancies, therefore, between the global and regional assessments, as different criteria are used and the way antimicrobials are used also varies in different parts of the world. The European Commission (EC) has issued guidelines regarding the prudent use of antimicrobials in veterinary medicine (EC, 2015) ideally to stop the use of antibiotics for routine prevention and only use them for metaphylaxis (control—USA) or treatment and to restrict the use of critically important antibiotics (CIAs) to situations where the veterinarian has assessed, on the basis of antimicrobial susceptibility testing and relevant epidemiological data that there is no noncritically important effective antimicrobial alternative available. The USA has followed a different approach, and in 2017, it has put antimicrobial use under veterinary control for those compounds considered of importance in human health and has stopped their use for growth promotion but allowed their use for prevention, control and treatment (FDA, 2012).
It is the purpose of this article to review the use of amoxicillin in swine, by assessing its pharmacodynamic and pharmacokinetic properties and available clinical data and to examine its position in relation to other critically important antimicrobial products.
2 MAIN PATHOGENS AND DISEASES IN SWINE
Some of the major bacterial diseases in pigs, against which amoxicillin is indicated, can be divided into three major categories (Taylor, 2013):
- Systemic infections—septicaemic/bacteraemic infections—which also primarily colonize the respiratory tract and tonsil—for example, Streptococcus suis, Haemophilus parasuis and Actinobacillus suis, as well as Erysipelothrix rhusiopathiae, which may colonize skin too.
- Respiratory infections—Pasteurella multocida, Actinobacillus pleuropneumoniae, Bordetella bronchiseptica, which can cause bacterial rhinitis, bronchitis and pneumonia.
- Enteric infections—Escherichia coli, Salmonella spp, the cause of enteritis and diarrhoea at various stages of production. Escherichia coli is also commonly associated with the mastitis, metritis, agalactiae (MMA) syndrome in sows after farrowing and appears to have a different susceptibility pattern and lower resistance when compared with the enteric isolates.
2.1 Systemic infections
The penam penicillins, including amoxicillin, are probably the most widely used antibiotics for the treatment and control of systemic/septicaemic infections in pigs, such as streptococcal meningitis, which affects 20%–30% of British herds, usually in the postweaning period (Taylor, 2013). Infection can be picked up in piglets from the sow as early as 5 days by aerosol, ingestion or contact, and invasion may take place via the tonsil. Bacteraemia may lead to septicaemia with localization in joints, meninges or heart valves, and pigs can become affected from 10 to 21 days of age. Classically, clinically sick pigs are injected with penicillins and the remainder of the group treated via the drinking water. This commonly leads to prophylactic/metaphylactic medication mainly delivered in the feed, during the anticipated period of risk, usually after weaning. Stresses and adverse environmental conditions can commonly precipitate the disease. The most common infection is caused by S. suis type 2, but there are approximately 35 serotypes, so commercial vaccination is difficult. Early joint infections from 10 to 14 days of age are caused, especially by S. suis type 1 and type 14 in Britain. Long-acting forms of ceftiofur (third-G cephalosporin), given at the time of processing (castration, teeth clipping, tail docking) in the first week of life, were particularly popular for prevention of infections in some countries, but its use is coming under more scrutiny due to the associated production of extended-spectrum beta-lactamase (ESBL) resistance genes and therefore has been voluntarily banned in some European Union (EU) Member States, like Denmark, the Netherlands and France.
Haemophilus parasuis (Glässer's disease) has many similarities, including colonization of the respiratory tract and invasion causing septicaemia and then lodging in joints, occasionally brain but more frequently serosal surfaces. It is widespread in herds but did not always cause major problems. With the advent of porcine reproductive and respiratory syndrome virus (PRRSV) and its immuno-depressive effect, especially on macrophages, the situation seemed to get worse. There are 15 serovars and many more unclassified but not all cause disease and not all are covered by commercial vaccines. Infection is likely by aerosol from the sow or infected roommates, but outbreaks are usually in young pigs 3–6 weeks of age and may also be associated with coughing and dyspnoea.
Actinobacillus suis is less widely reported and may be more common in North America. It has similar transmission and septicaemic spread but additionally, may cause skin lesions, pneumonic lesions similar to A. pleuropneumoniae and also more acute deaths in 1- to 6–week-old pigs. There are no commercially available vaccines for A. suis.
Erysipelothrix rhusiopathiae tends to affect older, grower pigs and is transmitted orally or via skin abrasions. In acute forms, it can be septicaemic, causing mortality, but in less severe forms is associated with classical red diamond lesions of the skin and can cause chronic damage to joints and the heart. There are two major serotypes, and vaccines are protective and are widely used in sows but less so in growing pigs.
2.2 Respiratory infections
Pasteurella multocida is considered an important agent able to cause respiratory infections usually in older pigs, following viral or especially Mycoplasma hyopneumoniae infections, resulting in secondary bacterial invasion and colonization (Taylor, 2013). Because of the common mycoplasma involvement, tetracyclines are the most commonly used antibiotic followed by the macrolides and finally fluoroquinolones, by injection. Amoxicillin alone can be successfully used for the secondary bacterial component.
Actinobacillus pleuropneumoniae can cause pneumonia on its own or again as part of the porcine respiratory disease complex (PRDC) involving viruses and mycoplasma infections. There are 15 serotypes and some commercial subunit vaccines can protect against them all. It causes classical necrotic lung lesions and pleurisy and can be fatal in acute cases. In many herds, there is a bout of coughing in growing pigs and recovery but leads to about 30% of pigs having lesions of pleurisy at slaughter and the more severely affected will incur penalties and condemnations. A variety of antibiotics are commonly used for both treatment and prevention, including amoxicillin.
Bordetella bronchiseptica is commonly associated with toxigenic P. multocida type D causing atrophic rhinitis in young piglets. This causes sneezing, but more seriously destruction of the turbinate bones and distortion of the nasal bones and may increase the chances of secondary bacterial pneumonia. Vaccines have proven particularly effective in preventing this condition.
2.3 Enteric infections
Escherichia coli can cause gut infections, which may lead to neonatal scours, septicaemia and death in very young piglets, 0–3 days of age (Taylor, 2013). Fluoroquinolones are often employed, either via oral dosers or by injection at this stage. Further diarrhoea and uneven growth can be seen at 7–10 days of age but the most common is after weaning in 90% of all herds. A number of factors contribute to the severity of the disease, which can result in death, diarrhoea and poor growth performance. A number of management factors, additives (zinc oxide), vaccines and also antibiotics can be used to mitigate the problem. The aminoglycosides, such as neomycin and apramycin and the polymyxin, colistin, are common antibiotic choices due to a low level of resistance. In contrast, there is a higher incidence of resistance to amoxicillin alone, but a much lower level of resistance when combined with clavulanic acid (see Pharmacodynamics section and Figure 1).
Salmonella enterica spp, especially S. Typhimurium, can occur in pigs causing deaths, diarrhoea and depressed growth. Again, the aminoglycosides and colistin are common antibiotic choices and amoxicillin plus clavulanic acid has proven successful in drinking water. In severe cases, individual pigs may require injections, following selection according to sensitivity testing. Generally, Salmonella spp control is via acidification of the drinking water or occasionally liquid feed, and vaccines have been used, if there are major clinical problems.
Pigs do face a number of potentially severe bacterial infections in early and later life and amoxicillin on its own plays an important role, especially against the septicaemic and respiratory bacterial infections, but to a lesser extent on its own for enteric infections due to high levels of resistance. Combinations with clavulanic acid are more effectively employed.
3 PHARMACODYNAMICS OF AMOXICILLIN
Penicillins or beta-lactam antibiotics act on the cell wall of bacteria preventing its formation. They inhibit the potency of the transpeptidase and other peptidoglycan-active enzymes called penicillin-binding proteins (Prescott, 2013). Amoxicillin, like other penicillins, is primarily considered a time-dependent bactericidal antibiotic once the concentration of the drug exceeds the minimum inhibitory concentration (MIC) of the organism. The individual bacterial species MICs of amoxicillin (El Garch et al., 2016; Marion & Siegwart, 2015; Yamamoto, Kijima, Yoshimura, & Takahashi, 2001) are summarized in Table 1.
Table 1. The amoxicillin MICs against a variety of bacteria—the number of isolates expressed as a percent
MIC values (μg/ml) |
≤0.03 |
0.06 |
0.12 |
0.25 |
0.5 |
1 |
2 |
4 |
8 |
16 |
32 |
≥64 |
Ref |
Bacteria |
No of isolates |
|
|
|
|
|
|
|
|
|
|
|
|
1 |
S. suis |
151 |
83.4 |
8.6 |
2.0 |
3.3 |
2.0 |
|
|
|
|
|
0.7 |
|
1 |
H. parasuis |
68 |
33.8 |
30.9 |
14.7 |
14.7 |
4.4 |
|
|
|
|
|
1.5 |
|
2 |
E. rhusiopathiae a |
214 |
15.9 |
38.8 |
42.5 |
2.8 |
|
|
|
|
|
|
|
|
1 |
P. multocida |
152 |
|
|
12.5 |
72.4 |
11.8 |
0.7 |
|
|
|
|
|
2.6 |
1 |
A. pleuropneumoniae |
158 |
|
|
3.2 |
57.6 |
27.8 |
|
|
0.6 |
|
0.6 |
0.6 |
9.5 |
1 |
B. bronchiseptica |
118 |
|
|
|
|
|
|
|
0.8 |
11.9 |
54.2 |
17.8 |
15.3 |
3 |
E. coli |
213 |
|
|
|
|
0.5 |
0.5 |
4.7 |
16.4 |
7.0 |
|
1.4 |
69.5 |
3 |
Salmonella spp |
127 |
|
|
|
|
|
28.3 |
3.1 |
0.8 |
|
|
|
67.7 |
- Refs: 1 = El Garch et al. (2016); 2 = Yamamoto et al. (2001); 3 = Marion and Siegwart (2015); MIC, minimum inhibitory concentrations.
For the potentially septicaemic bacteria, based on their susceptibility patterns, very little potential resistance was seen in S. suis (0.7% of isolates with high MICs) and H. parasuis (1.5%) against amoxicillin and no resistance was seen in E. rhusiopathiae against ampicillin, a similar amino-penicillin compound to amoxicillin. This aligned well with the proposed ≤0.5 μg/ml amoxicillin concentration for susceptible bacteria, 1.0 μg/ml for intermediate and ≥2.0 μg/ml for resistance interpretation by Schwarz et al. (2008). There were relatively clear visual demarcations of epidemiological cut-off values (ECOFFs) for these three bacteria. The Clinical & Laboratory Standards Institute (CLSI) ECOFFinder (2017) states that the visual ECOFF is generally similar to the 97.5% confidence figure that it produces. These ECOFF values are estimations of the “wild-type” MICs of the bacterial species before exposure to antimicrobials may have induced resistance. These must be differentiated from the clinical breakpoints (CBPs), which are related to the drug concentrations achieved at the site of infection, given by a specific route of administration and which have a positive clinical effect. They may be above or below the ECOFF depending on the particular antimicrobial. Richez, Keck, and Burren (2012) examined a further 65 French isolates of S. suis, to determine their MICs and also to look at a subset of six isolates likely minimum bactericidal concentration (MBC). All the MICs were in the range 0.004–0.15 μg/ml with a MIC 90 of 0.015 μg/ml. At the MIC, they were marginally bactericidal, but at two and four times the MIC, they were clearly bactericidal, on a time-dependent basis. No comparable individual isolate data were found for A. suis, but two reports described 9.4% resistance in the USA (Oliveira, 2006) and 13.3% resistance in Canada (Dufresne, 2006) both using ampicillin.
Regarding the respiratory bacteria, both P. multocida and A. pleuropneumoniae showed clear ECOFFs at 1.0 μg/ml and 0.5 μg/ml, respectively, and potentially resistance rates of 2.6% and 11.3%, respectively, above their ECOFFs (El Garch et al., 2016). Tanigawa and Sawada (2002) showed that there was a sub-MIC inhibitory effect at a quarter of the MIC for a particular strain of A. pleuropneumoniae, which disappeared when the amoxicillin was inactivated. At 1.25 times the MIC, there was a bactericidal effect seen even after 2 hours (hr). In contrast, B. bronchiseptica showed a completely different sensitivity pattern ranging between 4 and ≥64 μg/ml and was considered likely to be inherently resistant to amoxicillin (El Garch et al., 2016).
The enteric bacteria, E. coli and Salmonella spp, showed similar sensitivity patterns starting at 0.5 μg/ml with an ECOFF at about 16 and 8 μg/ml, respectively (Marion & Siegwart, 2015). High levels of potential amoxicillin resistance above the ECOFF were seen at 70.9% and 67.7%, respectively. The completely different susceptibility patterns suggest that there is a different resistance “driving force” in the gut associated with different pharmacokinetic factors, likely to be associated with direct oral administration of amoxicillin, giving high concentrations in the gut in comparison with plasma/blood and bronchiolar mucus/lung concentrations. Amoxicillin is excreted via the kidney and does concentrate in the urine. The CLSI (2008) does differentiate Streptococci and Enterobacteriaceae susceptibility MICs, using ampicillin, at ≤0.25 μg/ml and ≤8.0 μg/ml, respectively. Resistance is defined at ≥8.0 μg/ml and ≥32 μg/ml, respectively. The susceptibility of amoxicillin specifically against porcine bacterial strains has not been defined.
It appears that most of the resistance is associated with beta-lactamase production. When amoxicillin is combined with the beta-lactamase inhibitor, clavulanic acid, much of the resistance disappears (see Figure 1).
The ECOFF for amoxicillin against E. coli is 16 μg/ml. When clavulanic acid is added, then the level of resistance falls from 70.9% to 3.8% (see Table 2). This reduction in resistance effect can be seen with all the Gram-negative bacteria (El Garch et al., 2016) but not the Gram-positive S. suis, suggesting its resistance mechanism is other than by beta-lactamase production. For example, methicillin resistance, observed in Staphylococcus aureus and caused by the mecA gene, alters the penicillin-binding proteins in the cell wall and blocks all the beta-lactam antibiotics including amoxicillin.
In many respects, the aminobenzyl penicillins like amoxicillin could be classified along with penicillin, as amoxicillin is also susceptible to penicillinases, when separated out from combined use with beta-lactamase inhibitors. The combination product takes it into the same league as third-G cephalosporins for potency regarding resistance (El Garch et al., 2016). Clavulanic acid is also effective against the majority of extended-spectrum beta-lactamases (ESBLs), associated with third- and fourth-G cephalosporin resistance except the AmpC genes (Bush & Jacoby, 2010; EFSA, 2011).
Amoxicillin does show a different spectrum of activity in comparison with other antibacterials classed as critically important such as ceftiofur (third-G cephalosporin), enrofloxacin (fluoroquinolone), tilmicosin (macrolide) and tulathromycin (advanced macrolide—triamilide) (El Garch et al., 2016) against S. suis and A. pleuropneumoniae. Also there is a marked difference between amoxicillin and enrofloxacin and apramycin (aminoglycoside) against E. coli (Marion & Siegwart, 2015) (see Table 2). The ECOFF was determined from the visual pattern of MICs, and the percentage of isolates above the ECOFF was used as a measure of reduced susceptibility, as in not all cases have the specific clinical breakpoints for these antibiotics been determined.
Against S. suis, amoxicillin, the clavulanate combination, ceftiofur and enrofloxacin show a very high level of activity, with two percent or less of isolates above the ECOFF MICs. In contrast, tilmicosin showed that 66.9% of the isolates and tulathromycin 68.2% were above the ECOFF and likely to be resistant.
Against A. pleuropneumoniae, amoxicillin showed 11.3% of isolates were above the ECOFF whilst the clavulanate combination and tilmicosin and tulathromycin showed none, ceftiofur 0.6% and enrofloxacin 7.0%.
Escherichia coli showed a more varied pattern with 70.9% of isolates above the amoxicillin ECOFF, yet the combination with clavulanic acid only 3.8%. Enrofloxacin showed 27.2% above the ECOFF and apramycin 9.4%. In a different reference (European Food Safety Authority (EFSA), 2011), they reported only 2.3% of 1559 EU E. coli isolates from pigs were resistant to the third-G cephalosporin, cefotaxime, suggestive of extended-spectrum beta-lactamase (ESBL) production.
There are marked differences between the activities of the antibiotics against different species of bacteria and especially between amoxicillin on its own and in combination with clavulanic acid against E. coli, for example. From a responsible or prudent use perspective, only the combination with a beta-lactamase inhibitor really should be reserved for the critically important antimicrobial category along with third- and fourth-G cephalosporins and fluoroquinolones.
4 PHARMACOKINETICS OF AMOXICILLIN BY ROUTE OF ADMINISTRATION
Standardized approved amoxicillin dosing in pigs has been established for many years. Ready to use or preformulated, short-acting (SA) injectables are administered at 7 mg amoxicillin trihydrate/kg bodyweight once daily intramuscularly for up to 5 days (NOAH, 2016). Long-acting (LA) formulations were given at 15 mg amoxicillin/kg bodyweight intramuscularly and repeated after 48 hr. Both injectable formulations (SA & LA) were suspensions of 15% amoxicillin, as trihydrate, in different oil bases. The SA formulation's excipients include polysorbate 80, aluminium stearate and ethyl oleate, and the LA formulation's excipients are aluminium distearate and fractionated coconut oil. In drinking water, amoxicillin, as the trihydrate, is given at 16 mg/kg bodyweight (equivalent to 14 mg/kg bodyweight of amoxicillin) for 3–5 days and in feed at 15 mg amoxicillin/kg bodyweight daily for up to 14 days. In liquid feed as an oral powder, amoxicillin (as trihydrate) is approved at 20 mg/kg bodyweight for five consecutive days.
Agersø and Friis (1998a) examined the pharmacokinetics of SA and LA injection formulations of amoxicillin at 14.7 mg/kg and 14.1 mg/kg bodyweight, respectively, in comparison with an intravenous (IV) dose 8.6 mg/kg and also an oral formulation administered by gavage to pigs at 10.1 mg/kg bodyweight, which had been fasted and a group that had been fed to assess the impact of feeding on absorption of the compound. They used eight crossbred pigs, 30–50 kg bodyweight, in the two crossover designed studies comparing the IV results with the SA and LA injectable results in the first study and IV and fasted and nonfasted results in the second study. There was a 1-week washout period between each treatment for the four pigs in each group. The results are summarized in Table 3.
Table 3. Comparative pharmacokinetics of amoxicillin given intravenously, intramuscularly (SA & LA versions) and orally before feeding and after feeding (Agersø & Friis, ; Bes et al., )
PK parameter |
Intravenous |
Intramuscular (SA) |
Intramuscular (LA) |
Orally before feed (Bes results) |
Orally after feed |
Dose (mg/kg bwt) |
8.6 |
14.7 |
14.1 |
10.1 (20) |
10.1 |
Cmax (μg/ml) |
– |
5.1 |
1.7 |
1.6 (3.8) |
0.8 |
Tmax (hr) |
– |
2.0 |
1.3 |
1.9 (1.0) |
3.6 |
AUC0–∞ (μg hr/ml) |
23.5 |
33.1 |
47.6 |
6.5 (12.52) |
5.5 |
MRT (hr) |
1.5 |
8.8 |
66.8 |
8.7 |
10.4 |
F(%) |
100 |
82 |
111 |
31 |
25 |
- Cmax, Maximum concentration; Tmax, time to reach Cmax; AUC0-∞, area under the curve from dosing (0) to infinity; MRT, mean residence time; F, bioavailability; SA, short-acting formulation injection; LA, long-acting formulation injection.
The LA formulation had a lower maximum concentration (Cmax), a greater area under the curve to infinity (AUCo–∞) and mean residence time (MRT) than the SA formulation, which makes it more suitable for a time-dependent antibiotic. The oral administration had a marked reduction effect on AUC and bioavailability (F%), reducing it to 31% in comparison with amoxicillin given intravenously and intramuscularly and even further to 25% when given after feeding. Unfortunately, the pharmacokinetic graphs for the orally administered amoxicillin comparison were not published (Agersø & Friis, 1998a).
A report by Bes, Baleri, March, and Riera (1997), which did give the pharmacokinetic values when amoxicillin was administered orally at 20 mg/kg bodyweight, was used for comparison. The Agersø and Friis (1998a) results were quite similar to the Bes et al. (1997) results when adjusted for approximately double the dose, with the Cmax at 3.79 μg/ml, Tmax 1 hr, AUCo–∞ 12.52 μg hr/ml, and the Agersø and Friis (1998a) results Cmax at 1.6 μg/ml, Tmax 1.9 hr, AUCo–∞ 6.5 μg hr/ml using a microbiological assay rather than HPLC. It demonstrates the rapid reduction in amoxicillin plasma concentrations following oral administration, by almost 12 hr, in contrast to the more prolonged plasma concentrations seen after SA and especially LA amoxicillin use.
In both the oil-based injectables, plasma concentrations fall more slowly but the SA formulation, given even at double its approved dose, appears to go below the susceptible cut-off value of 0.5 μg/ml proposed by Schwarz et al. (2008) by 12 hr. The LA formulation appears to go below by 24 hr (Agersø & Friis, 1998a) (see Figure 2). Other LA formulations (Tanigawa & Sawada, 2002) lasted over 36 hr. Both the LA formulation's amoxicillin concentrations were above the MIC 90 for S. suis of 0.06 μg/ml for 48 hr, at 0.07 and 0.15 μg/ml, respectively.
A number of studies reported on the distribution of amoxicillin to other tissues or organs of potential relevance to the treatment of the different types of infection. Agersø and Friis (1998b) also compared plasma concentrations of amoxicillin with those found in lung and bronchial secretions, after IV injection at 8.6 mg/kg bodyweight. They found that AUC lung and bronchial secretion ratios against plasma AUC were 0.39:1 and 0.33:1, respectively, showing there was no concentration effect in lung tissue or bronchial secretions. The plasma protein binding (PPB) was 24% and in bronchial secretions 21% (Agersø & Friis, 1998b). Interestingly, in A. pleuropneumoniae-infected pigs, the PPB was reported lower at 17% and for bronchial secretions 13% (Agersø, Friis, & Nielsen, 1998). Palmer and Bywater (1978) reported that there was 40%–60% of serum concentrations found in joint fluid, 0.5–2 hr after treatment both orally and by injection, showing a reduced joint penetration. Agersø, Friis, and Haugegaard (1998) also reported on a study, where pigs were given a mean dose of 23 mg amoxicillin/kg bodyweight daily for 5 days in drinking water, and at this dose level, plasma concentrations reached a constant level between 0.5 and 1.3 μg/ml, at or above the proposed ≤0.5 μg/ml amoxicillin concentration for susceptible bacteria, by Schwarz et al. (2008).
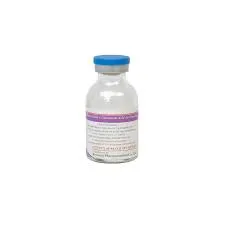
Amoxicillin and its main metabolite amoxicilloic acid are primarily excreted via the kidneys (Reyns, de Boever, de Baere, de Backer, & Croubels, 2008) hence its common use for the treatment of urinary tract infections. Only approximately 10%–14% is metabolized and excreted via the liver and therefore was considered likely to have relatively little activity on the gut flora. This was reported to not be the case by Bibbal et al. (2007) with ampicillin, a similar amino-penicillin, when administration by oral and IM routes at 20 mg/kg bodyweight for seven consecutive days increased the number of resistant Enterobacteriaceae and also E. coli. Using a blaTEM gene quantification method, they demonstrated that there was a difference between oral and injectable administration with the injectable being lower but still significantly higher than the untreated controls. The injection was given at a higher dose rate for a longer period than usual, which may have influenced the results. In a second study, Cavaco, Abatih, Aarestrup, and Guardabassi (2008) compared gut E. coli selection pressure using injections of amoxicillin, ceftiofur and cefquinome, given IM at 15, 3 and 3 mg/kg bodyweight, respectively, for three consecutive days. The pigs were inoculated intragastrically with 1010 colony-forming units (CFU) of a nalidixic acid (NA)-resistant mutant derived from a CTX-M-1 producing porcine E. coli. The total coliforms and CTX-resistant coliforms were counted, and the total resistant coliforms to NA and CTX, the original isolate, were also counted. Significantly higher counts of CTX-resistant coliforms were observed in the three treatment groups than in the controls over the 25-day trial period but ceftiofur and cefquinome exerted much larger selective effects than amoxicillin, especially in the first eight days after treatment started, when the amoxicillin group had lower CTX and NA+CTX-resistant coliforms than the untreated controls. There was a proliferation of indigenous CTX-M-producing strains by horizontal transfer in the pigs, which was considered responsible for the overall increase at day 25 in the amoxicillin group.
When given orally, the bioavailability of amoxicillin is between 25 and 31% depending on whether the pigs have been fed, and thus, substantial quantities pass along the gastrointestinal tract and may have a direct impact on the gut flora, especially E. coli. Some will be broken down in the gut by acids and enzymes, and Reyns et al. (2008) postulated that approximately 50% may be broken down presystemically due to higher amoxicilloic acid concentrations in the kidney and liver in comparison with amoxicillin given intravenously. However, E. coli in the gut will be exposed to potentially much higher concentrations of amoxicillin after oral administration and it is thought that this is why there is a high level of resistance seen in enteric E. coli and Salmonella spp. Using an antimicrobial model (Burch, 2012) estimated that amoxicillin administered in feed at 300 μg/g (to give approximately 15 mg/kg bodyweight), with an absorption of 30% might achieve concentrations of 357 μg/g in the faeces, providing there was no breakdown of active substance. Concentrations in the jejunum could be 107 μg/ml, again if there was no destruction of the active substance. Even if there was a 50% breakdown of the drug, concentrations achieved could be 54 μg/ml in the small intestine. Palmer and Bywater (1978) confirmed that therapeutically useful concentrations persisted in the stomach and small intestine until at least 12 hr after dosing but a figure was not reported. This oral exposure would account for the high MICs and high level of resistance found in enteric E. coli in pigs. Unfortunately, no published amoxicillin gut concentration data were available to confirm these findings, but the susceptibility pattern of E. coli supports this finding.
5 PK/PD CORRELATION
Amoxicillin is primarily considered a time-dependent bactericidal antibiotic once the concentration of the drug exceeds the minimum inhibitory concentration (MIC) of the organism. Orally administered penicillins have a very short half-life hence the requirement normally to receive multiple doses during the day, either in feed or drinking water to achieve a sufficient time above the MIC. The area under the concentration–time curve (AUC) and MIC can be used with compounds or formulations, which provide a more extended half-life, such as for the LA or SA formulations (Nielsen & Friberg, 2013), but it must be remembered that the AUC also has a time component.
The PK results from the Agersø and Friis (1998a) work were adjusted to fit the approved dosing of the SA formulation at 7 mg amoxicillin/kg bodyweight IM and the LA formulation of injection at 15 mg/kg bodyweight given IM and compared with the proposed cut-off of 0.5 μg/ml for systemic and respiratory infections and the MIC 90 of the highly susceptible S. suis at 0.06 μg/ml (see Figure 2).
The SA formulation's amoxicillin plasma concentration falls below the respiratory cut-off of 0.5 μg/ml at just approximately 9 hr. The required 40% time, above MIC for these bacteria, requires 9.6 hr. If the plasma protein binding (PPB) of 24% for healthy pigs is taken into account (Agersø & Friis, 1998b), then the amoxicillin effective plasma concentration will be less at approximately 6.8 hr or 28%, suggesting the dose might be too low for some of the respiratory infection bacteria, for example, P. multocida and A. pleuropneumoniae. Regarding the MIC 90 figure of 0.06 μg/ml for S. suis, the plasma amoxicillin concentration is above this figure for 23 hr or 96% and with PPB it is still 21 hr or 88% of time, well above the 40% time required. With regard to the LA formulation, there are two peaks reported, thought to be associated with the excipients (coconut oil and aluminium distearate) used in the formulation (Agersø & Friis, 1998a). Plasma concentrations fall below the cut-off of 0.5 μg/ml at approximately 19 hr or 79% of time, but if PPB of 24% (Agersø & Friis, 1998b) is taken into account, it is reduced to 10 hr or 42%, but it should be sufficient but for a daily injection for the respiratory bacteria. The PPB was reported to be slightly lower in A. pleuropneumoniae-infected pigs (Agersø, Friis, Nielsen, 1998) at 17%, but an explanation for this was not given. It is well above the MIC 90 for S. suis of 0.06 μg/ml for well beyond the 48 hr or (100%), so should be highly effective.
With regard to oral administration, the Bes et al. (1997) data were used. The original dose of 20 mg/kg bodyweight was halved to give a model of twice daily administration of 10 mg/kg bodyweight to be more in line with drug administration in water or feed. It was compared with the proposed cut-off of 0.5 μg/ml for systemic and respiratory infections and the MIC90 of the highly susceptible S. suis at 0.06 μg/ml (see Figure 3).
Regarding the sensitivity cut-off of 0.5 μg/ml for systemic and respiratory infections, the time above is approximately 9–10 hr or approximately correct but if PPB is taken into account, it is more like 8 hr (33%), which is potentially too short for some isolates. Regarding the MIC 90 for S. suis of 0.06 μg/ml the time above the MIC is 20 hr (83%) but with PPB of 24%, it is still 16 hr or 67%, so should be effective.
Oral administration is unlikely to be effective against systemic or septicaemic forms of E. coli infection, as the ECOFF is 16 μg/ml, but it is likely to be highly active against susceptible E. coli in the gut, due to direct exposure and against urinary tract infections, where amoxicillin is primarily excreted and concentrated.
Rey et al. (2014) took the PK/PD assessment for amoxicillin in pigs a step further, they determined the pharmacodynamic cut-offs using PK/PD principles and Monte Carlo simulations. A population model was developed for intramuscular and oral use, and they wanted to achieve a probability target attainment rate (PTA) of 90% for MICs between 0.0625 and 4.0 μg/ml for at least 40% of a 24 hr period. The PTA rate of 90% for IM injection at 15 mg/kg was achieved for a MIC of 0.0625 μg/ml, but a higher dose of 30 mg/kg bodyweight was required for a breakpoint of 0.125 μg/ml. A PTA rate of 90% was never achieved with the breakpoint recommended of 0.5 μg/ml (Schwarz et al., 2008) when administered at a single dose of 20 mg/kg bodyweight orally on a daily basis. They also divided the oral dose by two and gave it twice daily, but they could not achieve a PTA rate of >90% for 0.0625 μg/ml unless the daily dose was doubled to 40 mg/kg bodyweight.
Although using a different approach and possibly a higher PK assessment of a PTA rate of 90% rather than a mean value, which is 50% of a population, it did confirm that LA IM injection should be suitable at 15 mg/kg for the treatment of S. suis up to the MIC 90 but may be too low for the bacteria up to the ECOFFs of 0.5 μg/ml. In contrast, the oral administration at 20 mg/kg bodyweight was not suitable, when given in divided doses, but 40 mg/kg bodyweight was required for the MIC 90 of S. suis at 0.0625 μg/ml and was not sufficient for P. multocida and A. pleuropneumoniae. This predictive assessment was a cause for concern, but it did not look at actual clinical use and responses.
6 CLINICAL DATA AND FIELD EXPERIENCES
A number of studies have been reported, regarding the use of amoxicillin for various clinical infections.
6.1 Streptococcus suis
Krejci, Malasek, and Lopez (2013) reported on the use of a LA injectable formulation of amoxicillin administered prophylactically to piglets in farms with endemic S. suis infections. Approximately, 372 piglets were treated in the first 24 hr after birth with a single injection at 15 mg/kg bodyweight. Mortality was recorded on a daily basis, and it was noticeable that the main mortality was in the first 3 days of life. The untreated pigs and the treated pigs both showed marked reductions in mortality by day 4. The piglets had a mortality from day 1 to day 4 of 11.2% in the controls and 6.6% in the treated ones (−4.6%), and between day 1 and day 18 at weaning, there was a mortality of 14.98% in the controls and 9.68% (−5.3%) in the treated pigs. Richez, de Bruin, Burren, and van Nes (2012) reported on the use of a LA injectable formulation of amoxicillin for the treatment of piglets with S. suis arthritis causing lameness. However, it was shown that the piglets needed three or four daily IM injections at 15 mg/kg bodyweight to reduce lameness significantly and two doses were not sufficient, with relapses occurring 48 hr later. Thomas et al. (2002) compared the efficacy of amoxicillin injection with cefquinome (fourth-G cephalosporin) for the treatment of streptococcal meningitis. Fifty-five pigs, in each group, were treated IM with 2 mg/kg bodyweight of cefquinome or 7.5 mg/kg bodyweight of amoxicillin on a daily basis for 3–5 days. The pigs were selected when they were showing early signs of infection, such as elevated temperature, depressed demeanour, incoordination, paresis or paralysis, tremors or ataxia. At day 7, 51% of the cefquinome-treated pigs were cured and 47% of the amoxicillin pigs but 18% and 25% had died, respectively. By day 21, 67% of the cefquinome-treated pigs and 57% of the amoxicillin-treated pigs were cured but 24% and 33% had died, respectively. Meningitis was found in 28 of 29 pigs at necropsy. There were no untreated controls for ethical reasons. Of the piglets, which showed only one to three clinical signs, 88% survived, whereas those that had four to six clinical signs, only 54% survived. This trial highlights the potential severity of the disease and the requirement for prompt, early treatment.
6.2 Actinobacillus pleuropneumoniae
Agersø and Friis (1998a) showed that the LA formulation of injectable amoxicillin would give a sufficient plasma concentration to treat A. pleuropneumoniae in the first 24 hr of use. Agersø, Friis, Nielsen et al. (1998) demonstrated that in pigs, which had been artificially infected with A. pleuropneumoniae, the distribution of amoxicillin to bronchial secretions was increased compared with that previously reported for healthy pigs, while only minor changes in concentration were observed in lung tissue. Godoy et al. (2010) also confirmed amoxicillin plasma concentrations were also higher in respiratory disease affected pigs after oral administration of amoxicillin. This suggests that the PK results in healthy pigs might underestimate the plasma concentrations and the time above MICs achieved and thereby a better treatment effect might be seen. Tanigawa and Sawada (2002) administered a LA formulation of amoxicillin at 15 mg/kg bodyweight IM, 22 hr before the administration of A. pleuropneumoniae intratracheally to a small test group of three pigs. The MIC for the strain used in the study was 0.4 μg/ml. Another group was untreated but infected, and a third group was untreated and uninfected but in contact with the other pigs. The treated group of pigs showed only a mild level of disease in the 50-hr observation period postchallenge and no mortality, whereas all the untreated pigs in both infected and in contact died, demonstrating it was a severe challenge. Of interest, the serum concentrations for amoxicillin were above the MIC for 13 hr (54%) after challenge, which appears to be sufficient to prevent much of the challenge. In a second study, Tanigawa and Sawada (2003) challenged pigs with the same strain of A. pleuropneumoniae, as in the previous trial and when disease had developed after 6 hr five pigs/group were treated at 15 mg/kg, 7.5 mg/kg and 0 mg/kg bodyweight IM with a LA formulation of amoxicillin. Repeat injections were given after 48 hr if clinical signs remained. Mortality was zero in the treated groups after 7 days and 80% in the untreated controls. Lung lesion score was zero in the 15 mg/kg group, one in the 7.5 mg/kg group and seven in the untreated group and recovery of the isolate was zero, 20% and 100%, respectively. This dose of 15 mg/kg bodyweight of amoxicillin LA formulation showed a very positive treatment effect against an isolate with a MIC of 0.4 μg/ml. Kanzenbach and Lahrmann (2011) compared the macrolide tulathromycin given as a single dose of 2.5 mg/kg bodyweight IM and amoxicillin given in feed (to give 20 mg/kg bodyweight amoxicillin in two divided doses) for 7 days in the metaphylaxis of weaned pigs against an A. pleuropneumoniae field infection. Both drugs prevented an early infection. At the end of the growing period (7th week), an acute outbreak of A. pleuropneumoniae occurred and affected 95% of the amoxicillin pretreated pigs but not the tulathromycin-treated pigs. The oral administration of amoxicillin did not offer any prolonged protection from a recurrent A. pleuropneumoniae infection in comparison with tulathromycin given by injection. This could be due to the difference in their modes of action against A. pleuropneumoniae and the tulathromycin's induction of an immune response. Nienhoff (1998) compared a single crystalline free-acid preparation of ceftiofur given intramuscularly at 3, 5 and 7 mg/kg bodyweight IM and found it equally as effective as two injections of amoxicillin at 15 mg/kg bodyweight given IM given at a 48-hr interval, in treating pneumonia of mixed aetiology. The recommended dose of ceftiofur is 3 mg/kg bodyweight IM given daily for 3 days.
6.3 Escherichia coli
Jensen, Lykkesfeldt, Fryendahl, Møller, and Svendsen (2006) infected a group of recently weaned pigs with E. coli O149:F4 to induce diarrhoea, which it did 12–16 hr later. The piglets were then dosed with 20 mg/kg of amoxicillin by gastric gavage and the plasma PK of the diarrhoeic pigs was compared with the healthy ones. The Cmax observed in the healthy pigs was 7.5 μg/ml and in the infected pigs was 3.6 μg/ml (−52%), and the AUC0–24 hr was 46 and 28 μg hr/ml (−39%), respectively. This demonstrated that diarrhoea significantly reduced the absorption of orally administered amoxicillin. Agersø, Friis, and Nielsen (2000) showed by contrast that when pigs are infected with Salmonella enterica Typhimurium and then injected with 15 mg/kg bodyweight of amoxicillin IM, 24 hr after infection, the AUCo–∞ was 35 μg hr/ml in the healthy pigs but even higher at 54 μg hr/ml in the infected pigs (+54%) although this was not significant. The MRT was also elevated from 6.3 to 17 hr, respectively, and was significantly different. However, the Cmax was higher in the healthy pigs at 4.7 μg/ml than in the infected pigs at 3.7 μg/ml (−21%), and the Tmax was 2.1 hr in healthy pigs but 1.3 hr in infected pigs, although not significantly different. The authors reported that inoculation with S. Typhimurium resulted in a change in plasma concentration–time profile, characterized by significantly faster absorption from the injection site, with the absorption rate constant being significantly higher at 3.1 in comparison with 1.04 in healthy pigs. It was postulated that it might be caused by higher body temperatures and shivering and diversion of blood supply to the muscle tissues. It was thought that the excretion was slower due to the infection, with the elimination half-life increasing from 3.6 hr in healthy pigs to 11.2 hr in infected pigs. It was thought that it may be linked to the increase in volume of distribution, again related to muscular blood flow. Distribution of amoxicillin to the intestinal tract tissue was only affected to a minor degree. Unfortunately, neither paper reported on the concentration of amoxicillin in the gut contents, or the response to treatment. Heinritzi & Hagn, 1999; reported on the comparative efficacy of cefquinome (fourth-G cephalosporin) given at 1, 2 and 4 mg/kg bodyweight IM and amoxicillin SA given at 7 mg/kg bodyweight IM to sows for three consecutive days for the treatment of puerperal septicaemia and toxaemia syndrome. In 41% of sows, endometritis was the only infection and 72.3% of those sampled were E. coli. In 70% of cases, mastitis was found with a mixed infection, streptococci and micrococci were also involved. Cefquinome at 2 and 4 mg/kg bodyweight were clearly more effective than cefquinome at 1 mg/kg bodyweight and 7 mg/kg bodyweight of SA amoxicillin. No statistical evaluation was reported to help clarify the results. It is possibly not surprising that amoxicillin injection was not so effective, owing to the heavy involvement of E. coli. Thomas et al. (2000) compared marbofloxacin (fluoroquinolone) at 2 mg kg−1 day−1 IM for 3 days with amoxicillin SA at 7.5 mg/kg bodyweight IM for 3 days in the treatment of mastitis, metritis and agalactiae (MMA) syndrome in sows. The clinical response to sows was higher in the marbofloxacin group, especially at day 4, with a clinical response rate of 41% success, 59% improvement and 0% failure rates in comparison with amoxicillin injection 34% success, 49% improvement and 17% failure. The most commonly cultured pathogen was E. coli (48.1%) with an MIC 90 for marbofloxacin of 0.21 μg/ml and 32.3% resistant to amoxicillin. Streptococci (36.9%) and staphylococci (12%) were also found. The amount of E. coli resistance to amoxicillin would account for almost all of the treatment failures (15.5% of the 17% failures).
The clinical trial results show a number of interesting factors regarding the use of amoxicillin in pigs. Firstly, clinical disease can have quite a marked effect on PK values, with A. pleuropneumoniae infections delaying absorption but increasing bronchial secretions (Agersø, , Friis, Nielsen (1998), when given by injection but increasing absorption and achieving a higher Cmax and AUC for orally administered amoxicillin (Godoy et al., 2010). In contrast, enteric infections reduce absorption of amoxicillin from the gut, possibly associated with increased gut flow and therefore reduce plasma concentrations (Jensen et al., 2006). Unfortunately, gut concentrations were not monitored but these are essential for good gut microbe control and PK/PD correlation against organisms that bind on the surface of the gut such as E. coli. Concentrations in the mucosa or gut wall specifically relate to bacteria that live inside the cell such as Lawsonia intracellularis infections. The degree of mortality that can be seen with some of the infections, such as streptococcal meningitis and pleuropneumonia, highlights the severity of some of the conditions that have to be faced. Generally, LA amoxicillin injections at 15 mg/kg bodyweight gave good results for the treatment and prevention of S. suis and A. pleuropneumoniae infections but only moderate results regarding E. coli infections affecting sows postpartum. Amoxicillin given in feed at 20 mg/kg bodyweight were highly effective in controlling A. pleuropneumoniae infections. High levels of resistance in E. coli did have an impact on amoxicillin efficacy, in comparison with cefquinome (fourth-G cephalosporin) and marbofloxacin; however, these are two of the more critically important antimicrobial families in human medicine.
7 CURRENT EU PERSPECTIVES
The EU is in the process of increasing the control of the use of antimicrobials in veterinary medicine used both in feed (EC, 2014a) and by water and injection (EC, 2014b) in an attempt to try to reduce the use of antimicrobials, especially the critically important ones for humans. Prophylactic use is coming under much scrutiny and is likely to be not allowed, except in exceptional cases. Metaphylactic use is likely to be permitted, which is essential in population medicine when dealing with a number of enzootic porcine infections like S. suis and A. pleuropneumoniae, where the infections can be very severe and mortality can be very high, as reported in several trials included in this review.
The classification or categorization of the various antimicrobial families is ongoing in the EU, and based on the antibiotic susceptibility reported here and the methods of resistance development, there is some rationale to separate amoxicillin and other aminopenicillins alone, from combined use with clavulanic acid and other beta-lactamase inhibitors. The beta-lactamase inhibitors solve many of the penicillinase and other beta-lactamase resistance issues, including ESBLs except AmpC, that affect amoxicillin and it is only the combination, which makes it equal in activity terms to the third-G and fourth-G cephalosporins and other more critically important antimicrobials. Much has been prepared of PK/PD assessments of use of amoxicillin and the potential underdosing that occurs especially after Monte Carlo simulations (Rey et al., 2014), but the clinical effect of the LA injection form at 15 mg/kg bodyweight and in feed oral application at 20 mg/kg bodyweight in divided doses against S. suis and A. pleuropneumoniae suggests otherwise, unless resistance is an issue. This may be due to the altered PK values when animals are diseased rather than healthy, or the impact of sub-MIC effects. It may be misleading to pursue changes in dose just on PK/PD principles, without taking into account clinical responses, pharmacovigilance and lack of efficacy reports. A more complete assessment is required, before finite recommendations are implemented regarding dose and indication.
8 FUTURE PERSPECTIVES
Amoxicillin is an important antibiotic in porcine and human medicine. It is highly effective against several severe, acute onset and common systemic infections (e.g., S. suis, H. parasuis, E. rhusiopathiae) and respiratory diseases (e.g., A. pleuropneumoniae, P. multocida), against which resistance has not become a major problem to date. It is important that it remains available as a veterinary medicine but under responsible use considerations, such as after laboratory diagnosis, culture and sensitivity testing. Amoxicillin alone may be differentiated in the future from the combination product including clavulanic acid in classification terms. This might enable amoxicillin to be used before the more critically important antimicrobials, such as the third-G and fourth-G cephalosporins, fluoroquinolones and possibly the macrolides and triamilides. Combinations with clavulanic acid can be kept in reserve and used when antimicrobial resistance is an issue and is positively identified by culture and sensitivity testing. This appears to be primarily associated with enteric infections (e.g., E. coli and Salmonella spp) and where aminoglycosides and polymyxins are more widely used anyway. Early treatment/ metaphylaxis must be retained to obtain a positive treatment effect and protect the health and welfare of the animals under the care of veterinarians, S. suis meningitis and A. pleuropneumoniae infections are prime examples. Further PK/PD work based on population medicine is likely to be carried out but the PK changes due to clinical disease and also positive clinical results should be included in the assessment, before dosing changes are recommended to or implemented by the regulatory authorities.
9 AUTHOR CONTRIBUTIONs
DGSB prepared the original draft of the manuscript, and DS reviewed, amended and advised on subsequent drafts. Both authors have read and approved the final manuscript.